Science behind the Q Therapeutic System
Optimizing the Brain's Learning Rate
Learning in the brain takes many forms, all of which center around the synchronous firing of neurons within a network of shared function (or neuronal network). Each network has a unique electrical pattern, which plays a crucial role in relearning.
The brain's learning rate is a critical factor in the learning process. Learning rate can refer to the speed and efficiency with which neural networks adapt to new information or recover from damage, such as after a stroke.
BRAIN.Q's investigational therapy aims to optimize the brain's learning rate following a stroke, enhancing its ability to adapt and heal. This is done by exposing damaged neural networks to oscillating fields similar to those in a
healthy brain.
Biological Basis for BRAIN.Q Therapy
Recent evidence suggests that sustained exposure to these oscillating fields might change the oxidative stress balance, leading to changes in gene expression and restored homeostasis in the brain. In this way, BRAIN.Q therapy aims to trigger a dual process of neuroprotection and neurorecovery that helps restore and rebalance network dynamics, aiding in faster and improved brain relearning and recovery following a stroke.
Learn more about the science behind what we do
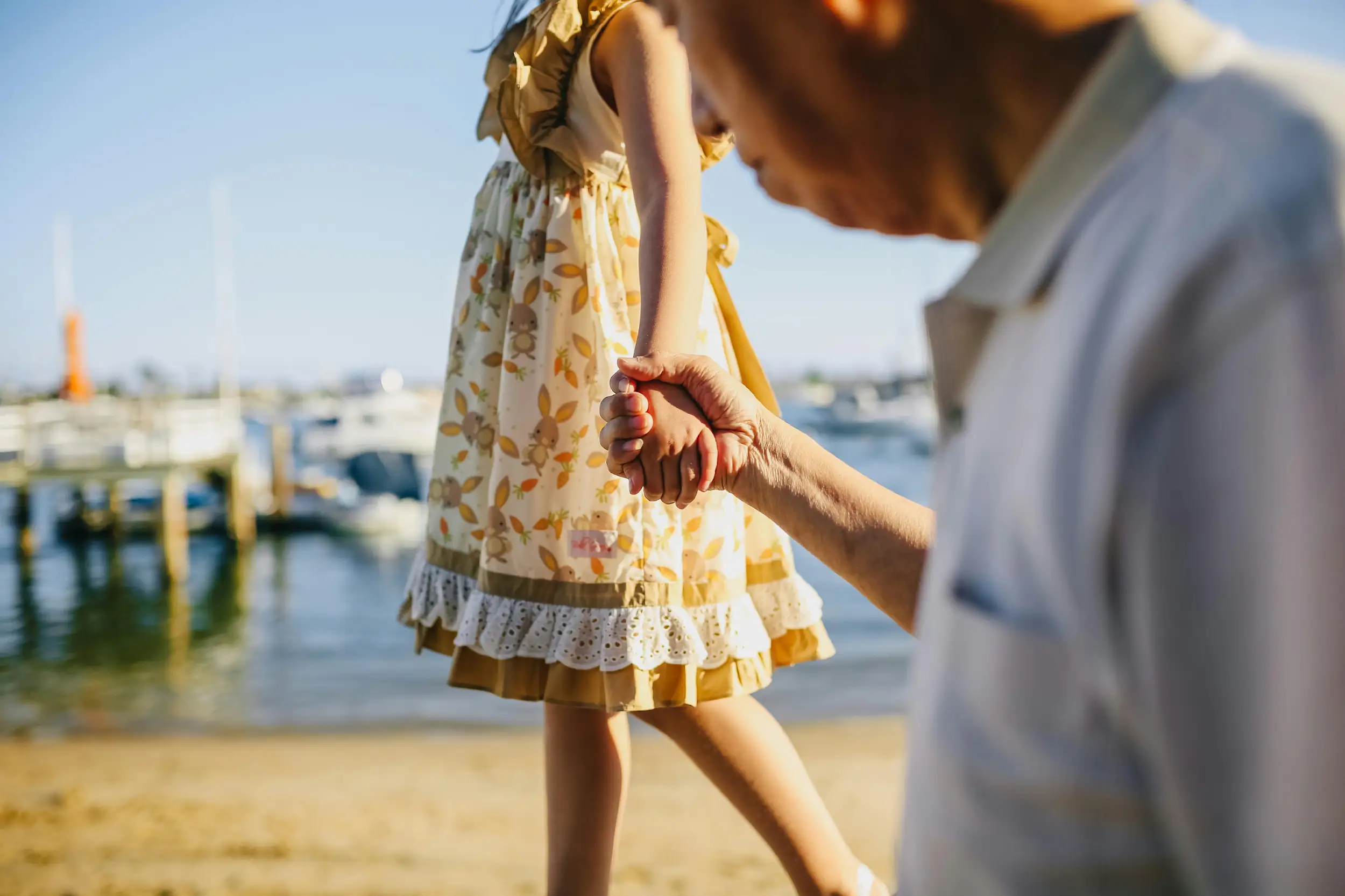
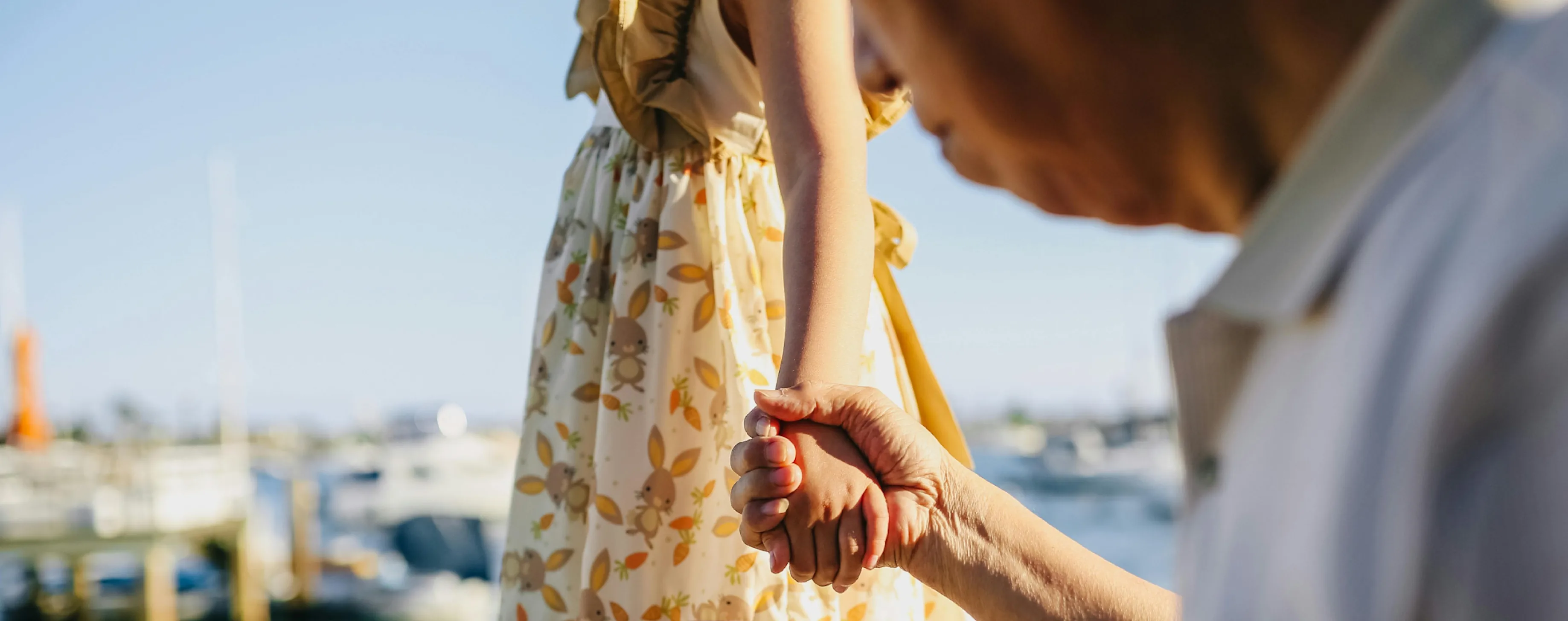
Mechanism of Action:
Click here to download our white paper explaining the science behind the Q Therapeutic System
Pilot Study Results
Weisinger et al., 2022
*ENTF refers to Electromagnetic Network Targeting Field [BRAIN.Q Therapy]
EMAGINE Trial Results
Saver et al, 2024, European Stroke Conference (ESOC) Late Breaking Science Plenary Session
BRAIN.Q is dedicated to providing evidence-based therapy through rigorous scientific research
Learn more about our ongoing EMAGINE.II Trial (NCT06386874)
Publications
Balter, S., Doniger, G., Weisinger, B., Bornstein, N., Shohami, E. Segal, Y., Alter, A., Lifshitz, A., Prasad, A. & Pandey, D. EEG Signatures of Frequency-Tuned Non-invasive Brain Stimulation over the Course of Stroke Recovery. American Clinical Neurophysiology Society (ACNS) Annual Meeting 2022
Balter Sr, Doniger G, Weisinger B, Segal Y, Shohami E, Lifshitz A, Pandey D, Alter A, Prasad A, Bornstein N. Delta Asymmetry And Relative Power Over Affected Hemisphere Correlates With Clinical Improvement (Mrs, Nihss) During The Course Of Post-Stroke Frequency-Tuned ELF-EMF Intervention. International Journal Of Stroke 2022 Oct 1 (Vol. 17, No. 3_ Suppl, Pp. 120-121).
Okabe N, Hovanesyan M, Azarapetian S, Dai W, Weisinger B, Parabucki A, Balter SR, Shohami E, Segal Y, Carmichael ST. Theta Frequency Electromagnetic Stimulation Enhances Functional Recovery After Stroke. Transl Stroke Res. 2023 Nov 14. doi: 10.1007/s12975-023-01202-z. Epub ahead of print. PMID: 37962771.
Saver JL, Duncan PW, Stein J, Cramer SC, Eng JJ, Lifshitz A, Hochberg A, Bornstein NM. EMAGINE-Study protocol of a randomized controlled trial for determining the efficacy of a frequency tuned electromagnetic field treatment in facilitating recovery within the subacute phase following ischemic stroke. Front Neurol. 2023 May 5;14:1148074. doi: 10.3389/fneur.2023.1148074. PMID: 37213907; PMCID: PMC10196621.
Segal Y, Segal L, Blumenfeld-Katzir T, Sasson E, Poliansky V, Loeb E, Levy A, Alter A, Bregman N. The Effect of Electromagnetic Field Treatment on Recovery from Ischemic Stroke in a Rat Stroke Model: Clinical, Imaging, and Pathological Findings. Stroke Res Treat. 2016;2016:6941946. doi: 10.1155/2016/6941946. Epub 2016 Feb 1. PMID: 26949561; PMCID: PMC4753339.
Segal Y, Segal L, Shohami E, Sasson E, Blumenfeld-Katzir T, Cohen A, Levy A, Alter A. The effect of electromagnetic field treatment on recovery from spinal cord injury in a rat model–clinical and imaging findings. Int J Neurorehabilitation. 2016 Apr;3(1000203):2376-0281.
Weisinger B, Pandey DP, Saver JL, Hochberg A, Bitton A, Doniger GM, Lifshitz A, Vardi O, Shohami E, Segal Y, Reznik Balter S, Djemal Kay Y, Alter A, Prasad A, Bornstein NM. Frequency-tuned electromagnetic field therapy improves post-stroke motor function: A pilot randomized controlled trial. Front Neurol. 2022 Nov 14;13:1004677. doi: 10.3389/fneur.2022.1004677. PMID: 36452175; PMCID: PMC9702345.
Weisinger, B. S., Bornstein, N. M., Shohami, E., Segal, Y., Alter, A., Lifshitz, A., Prasad, A., & Pandey, D. (2021). Abstract P194: Artificial intelligence-powered non-invasive and frequency-tuned electromagnetic field therapy improves upper extremity motor function in sub-acute stroke patients: A pilot randomized controlled trial. Stroke, 52(Suppl_1), AP194. https://doi.org/10.1161/str.52.suppl_1.P194